The Winding Road Toward Zero-Carbon Iron
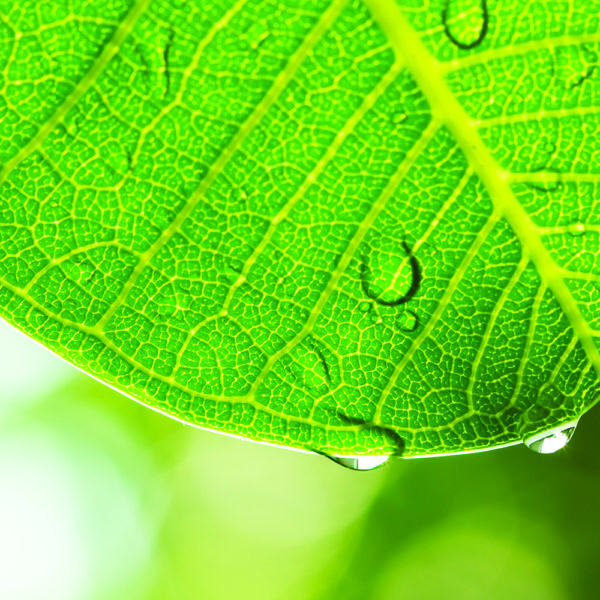
INTRODUCTION
The steel industry’s biggest product today isn’t steel — it’s carbon
Iron and steel production is responsible for about 9% of global CO2 emissions. A decisive shift away from carbon-based reductants to alternatives like hydrogen is all but inevitable – but it will have to come in stages.
Roughly 1.8 tons of CO2 are released into the atmosphere for every ton of liquid steel produced by the still predominant Blast Furnace/Basic Oxygen Furnace (BF/BOF) route – a number based on calculations that assume an average modern BF operated in OECD Europe. However, there are many plants around the world that emit two or more tons of CO2 per ton of steel. These facts are hardly good news to decision makers inside the industry. But they are only beginning to dawn on governments around the world that are now weighing their options for achieving the massive reductions in greenhouse gas emissions (GHG) mandated by the Paris Climate Agreement. As some of these governments strive toward the even more ambitious goal of net-zero carbon emissions by 2050, scrutiny is inevitably going to increase, and pressure is going to build on steel producers to bring down emissions in drastic ways. Emissions trading schemes are going to be implemented and widened in scope, carbon taxes are looming, and consumers will ultimately show concern for the carbon footprint of steel products.
Producers in some parts of the world are already feeling the painful pinch of carbon pricing. The development of prices for European CO2 Emissions Allowances, as illustrated in Figure 1, is a prime example. At their peak in mid-2021, prices had seen close to an eight to tenfold increase over a four-year period. And they remain near record highs, as the EU emissions trading scheme entered its next phase in 2021, when several measures kicked in that will progressively tighten the supply of allowances.
In anticipation of such regulatory measures and market pressures, steel producers around the world are racing to deploy new technologies aimed at reducing carbon intensity in iron and steelmaking. Merely shifting iron production from coal and coke-based blast furnaces to natural gas-based direct reduction plants will not be sufficient. The industry will need to develop other energy sources without a direct carbon footprint, such as hydrogen based on renewable energy to commercial scale and in a manner that is economically feasible.
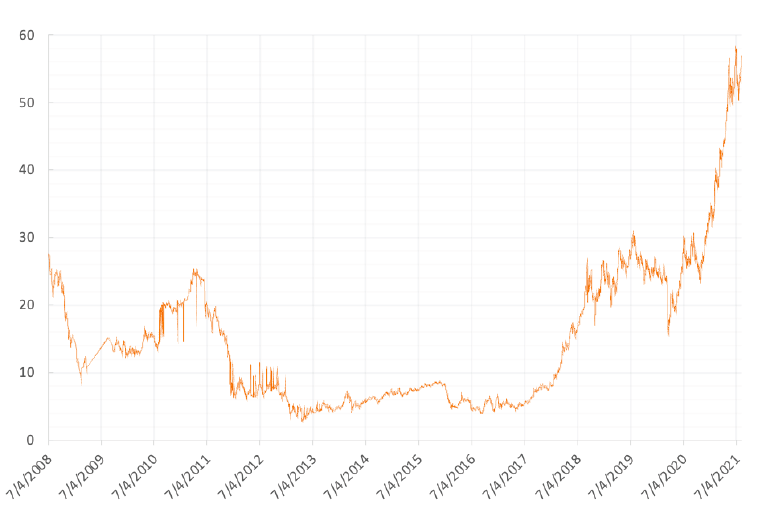
FIGURE 1. Price-Tag Increases for Carbon in the EU (EUA) (www.eex.de)
After hovering at low levels for years, the price of European CO2 Emissions Allowances has recently skyrocketed. As emissions-trading schemes are set to spread, allowances will inevitably become a significant driver of cost.
COMPARING THE PRODUCTION ROUTES
As Figure 2 shows, the Direct Reduction/ Electric Arc Furnace (DR-EAF) route, using natural gas in the DR process, cuts carbon intensity for liquid steel by more than 50% in comparison with the conventional BF/BOF route. By using “green” hydrogen instead, emissions can be reduced by 85-90%.
Most emissions at this point are actually attributable to electricity production for the EAF process. The calculations for Figure 2 are based on a CO2 emission grid factor of 0.226 kg of CO2 per kWh, which is the average value for EU- 27 from 2019. Applying the CO2 emission grid factor of Sweden (currently at 0.023 kg of CO2 per kWh) would bring emissions down to only 181 kg CO2 per ton — a whopping 90% reduction compared to the BF/ BOF route. From there, all that stands in the way of true zero-carbon iron would be the provision of fossil-free energy for electricity, heat, and transport.
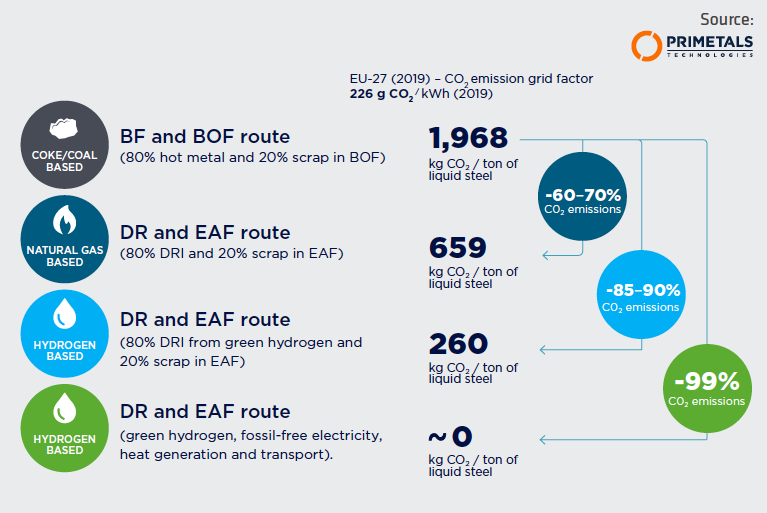
FIGURE 2.
CO2 emissions of different process routes to liquid steel (CO2 emission grid factor 0.226 kg/kWh – EU27 2019)
PRODUCING HYDROGEN AT SCALE
Needless to say, establishing a ready supply of hydrogen that can make iron and steelmaking truly carbon-free will be a major challenge. One of the key barriers is the sheer hydrogen volume needed to support a massive upscaling in use by the industry. The amount that will be consumed is tremendous. Converting a typical integrated plant with a production of 5 million tons per year from coal and coke to hydrogen will require a supply of at least 480,000 Nm³ (equaling 44 tons) of hydrogen per hour. To put this into perspective, the largest proton exchange membrane (PEM) electrolyzer currently in operation generates only about 3,000 Nm³ (i.e., 0.25 ton) of hydrogen per hour.
Not only will vast quantities of additional hydrogen be needed to support the steel industry, but it will also have to be produced using alternative processes. Currently, around 95% of hydrogen is “gray” – meaning it is produced by extracting gas from fossil fuels. It is possible to use carbon capture, utilization, and storage (CCUS) technology to prevent emissions from being released into the atmosphere, resulting in “blue” hydrogen. But this only makes economic sense where a high volume of CO2 can be captured at a single site. And it is only feasible in areas where there are geologically safe places to store captured carbon, such as beneath the sea or deep underground.
HYDROGEN ELECTROLYSIS WITH “GREEN” ENERGY
As of today, most of the hydrogen for industrial use is produced by steam methane reformers (SMRs). Since the natural gas that feeds these reformers (methane – CH4) contains carbon, the resulting “gray” hydrogen causes a sizable amount of CO2 emissions. To fully decarbonize the process, hydrogen for ironmaking will have to be produced by electrolysis from water, using fossil-free energy.
Since the year 2000, more than 230 hydrogen-electrolysis projects (counting both those based on renewable, as well as conventional sources of energy) have entered into operation around the world. Most of them, like the H2Future project are based in Europe. But several have been started or announced also in Australia, China, and the Americas. Almost all have been at a scale of less than 10 MW, but a 20 MW plant is now operating in Canada and lately there have been several proposals for plants exceeding 100 MW.
There are three main electrolysis processes that make all of this possible: alkaline, proton-exchange membrane (PEM), and high-temperature steam electrolysis. The most advanced technology at this point—and the one that most recent hydrogen-generation projects have favored, is Proton-Exchange Membrane or PEM (Figure 3). It attaches electrodes on two sides of a solid polymer membrane, which act as the electrolyte and as a separator to prevent the produced gases from mixing. Hydrogen ions form at the anode, pass through the membrane, and combine with electrons from the cathode to form hydrogen gas.
The PEM-type electrolyzer has several advantages: It is highly efficient, features high power density, and has an extended dynamic operating range, allowing it to be directly coupled to renewable sources—because it can quickly react to changes in the electricity supply. Modules are available in the range of 3 to 100 MW, producing up to 20,000 Nm³ (or roughly 2 tons) of hydrogen per hour.
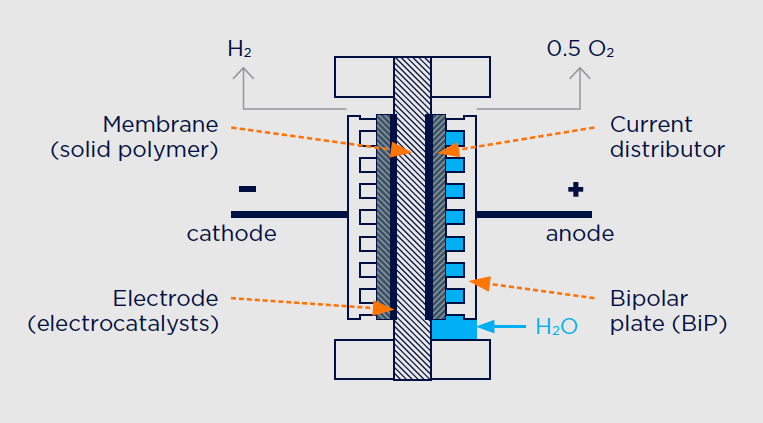
FIGURE 3.
Working principle of a proton-exchange membrane (PEM) electrolysis cell.
APPLYING HYDROGEN IN COMMERCIALLY PROVEN DIRECT REDUCTION SOLUTIONS
Hydrogen (H2) is already part of the reduction gas mix in mainstream direct reduction processes—alongside carbon monoxide (CO), of course. This flexibility of direct reduction processes provides an enticing way for plant owners to switch to hydrogen in a gradual fashion, increasing the addition of hydrogen over time as prices come down and supplies increase. It also underlines the value of new investment in direct reduction plants: Whatever the future might hold in terms of emissions regulations or prices for raw materials, direct reduction technology allows for maximum adaptability.
HYDROGEN AND THE MIDREX PROCESS
MIDREX® Direct Reduction Plants produce roughly 60% of the world’s DRI and have been available from Primetals Technologies for more than 30 years. The reducing gas – mainly a mixture of H2 and CO – is produced from natural gas in a special CO2 reformer. Without any modifications to the equipment, the process allows up to 30% of the natural gas to be replaced by hydrogen (Figure 4). For example, 60,000 Nm³/h of hydrogen could be brought in to substitute 20,000 Nm³/h of natural gas. With minor additions to the equipment (to protect the reformer), the rate can reach as high as 100% – the limit determined by the required carbon content in the final product. The process can easily accommodate fluctuations in the hydrogen addition rate, allowing the plant to react to changing hydrogen supplies that are to be expected when sourcing the gas from water electrolysis with renewables like wind or solar.
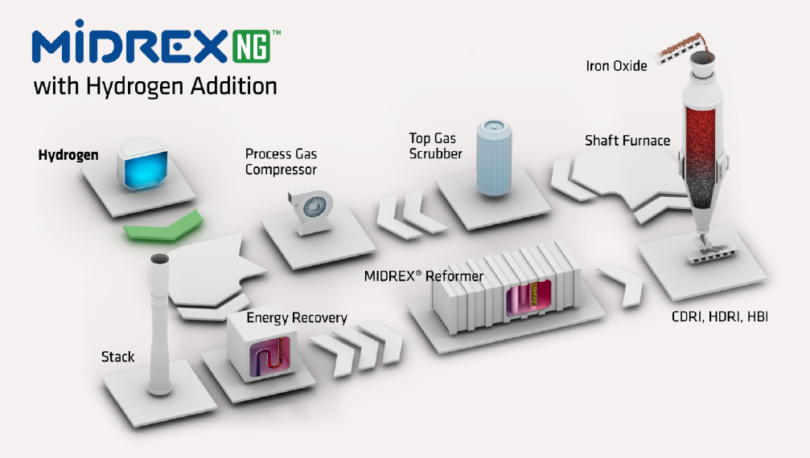
FIGURE 4.
MIDREX NG with Hydrogen Addition
MIDREX ON 100% HYDROGEN
If hydrogen is to be used as the sole reductant (MIDREX H2 ™ configuration), the natural gas reformer can be replaced by a reduction gas heater (Figure 5). Hydrogen will be converted to H2O during reduction and condensed in the top gas scrubber. Since there is no source of CO in the process loop, there is no need for a CO2 removal system. The process uses approximately 650 Nm³ of hydrogen per ton of DRI for reduction. Additionally, it requires about 250 Nm³ of H2 per ton of DRI for heat, which can be accommodated also by other energy sources (e.g., electrical energy). Of course, in case a flexible utilization with hydrogen (0 – 100% hydrogen) and natural gas is required from the beginning, the plant can also be designed in such way to meet this demand as well.
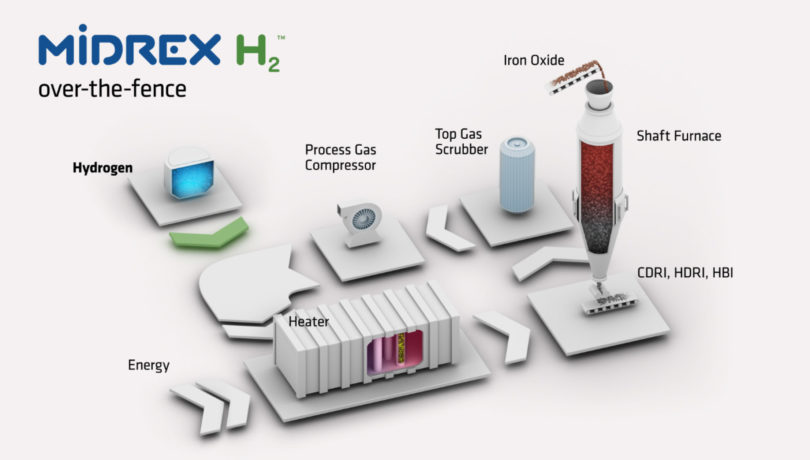
FIGURE 5.
MIDREX H2 with 100% hydrogen (The reformer can be replaced by a heater, which can be powered by carbon-free energy)
CONCLUSION – A “GREEN” HYDROGEN REVOLUTION
To be a true game changer, the hydrogen used in the ironmaking process must be “green” hydrogen, generated by electrolysis from water, using fossil-free power only. To date, producing hydrogen in this way has proven too costly to be competitive. But this is changing, as the boom in renewable energy sources, such as wind and solar power, brings down global electricity prices and carbon capture, utilization, and storage (CCUS) technology offers a way to store energy in times of excess electricity production, i.e., when the wind is blowing, or the sun is shining during times of low demand. Establishing a policy and financial incentive framework for adopting hydrogen is an essential part of this shift toward a more sustainable future – one which gives industry leaders the confidence to invest in long-term hydrogen projects.
Hydrogen is already part of the reduction gas mix in mainstream direct reduction processes. The flexibility of direct reduction processes provides an enticing way for plant owners to switch to hydrogen in a gradual fashion, increasing the addition of hydrogen over time as prices come down and supplies increase. It also underlines the value of new investment in direct reduction plants: Whatever the future might hold in terms of emissions regulations or prices for raw materials, direct reduction technology allows for maximum adaptability.
Using hydrogen to decarbonize ironmaking and other industrial processes is a powerful idea but not actually a new one. Leaders in the industry who are now nearing retirement may remember it vividly from their student days. There were peaks of interest in the 1970s during the oil price shocks, the 1990s with incipient concerns about climate change, and in the early 2000s on the same note. None of these developments resulted in a breakthrough moment for hydrogen. However, this time around may well be different, as the International Energy Agency (IEA) noted in a recent report, “last year marked a period of unprecedented momentum for hydrogen, combining a new depth of political enthusiasm with a breadth of new possibilities around the world.”
Article was adapted from articles originally published in Primetals Metals Magazine, January 2020, with supplemental figures supplied by Midrex Technologies.